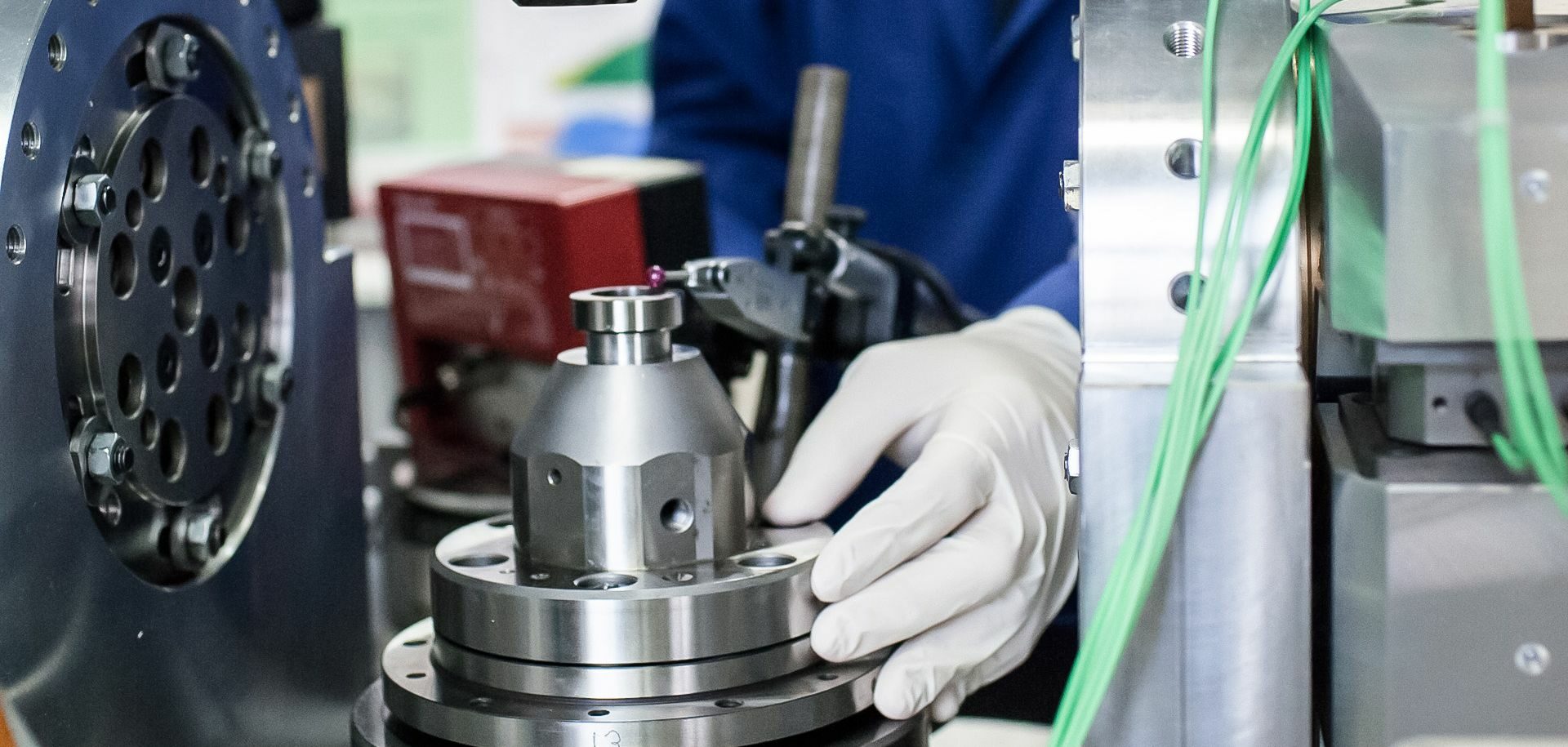
From lubricant physics to bearing behaviour
The SKF Chair at LaMCoS – INSA Lyon: "Lubricated Interfaces for the Future”.
Lubricants and their thermo-physical properties play a central role in research that tackles new problems related to both the evolving operating conditions of rolling element bearings and the response of the lubricant applied to them. These aspects are being studied at LaMCoS – INSA Lyon in cooperation with SKF.
In the mid-1990s, the SKF European Research Centre (SKF ERC, now Research & Technology Development, SKF-RTD) and the Laboratoire de Mécanique des Contacts (LMC, now Laboratoire de Mécanique des Contacts et des Structures, LaMCoS) at INSA Lyon began a collaboration on the lubrication of flange/roller-end contacts such as those found in large-size roller bearings. The key objective was to develop a new test bench, Tribogyr, dedicated to types of lubricated contacts that were barely considered in the scientific literature at that time. The unique specifications formulated by SKF included:
- simulate, on a 1:1 scale, a real contact such as those found in roller bearings with outside diameters of 600 to 1,000 mm,
- reproduce the particular kinematics that can be found in these contacts, with an entrainment velocity and also with spin and skew components,
- respect the radii of curvature of the rolling bodies in the contact area, and
- measure the three forces and the torque acting on each of the two specimens.
When Tribogyr was validated and put into service, limitations quickly appeared when comparing the experimental results with simulations that allowed the scope of investigation to be extended and the lubricant to be artificially changed. Other approaches were gradually introduced in the projects on the study of large-size lubricated contacts with spinning using Tribogyr (see http://evolution.skf.com/the-beast-of-lyon-putting-large-bearing-contacts-to-the-test/). This research, which was mainly motivated by SKF, became part of a more general research framework that was previously established at LaMCoS and named “A quantitative multi-physics, multi-scale and multi-approach to lubrication and lubricants”.
This general framework is first presented here with some illustrations that refer to the flange/roller-end contacts. More specific aspects are detailed afterwards, still in connection with the lubrication of heavily loaded contacts or elastohydrodynamic lubrication (EHL).
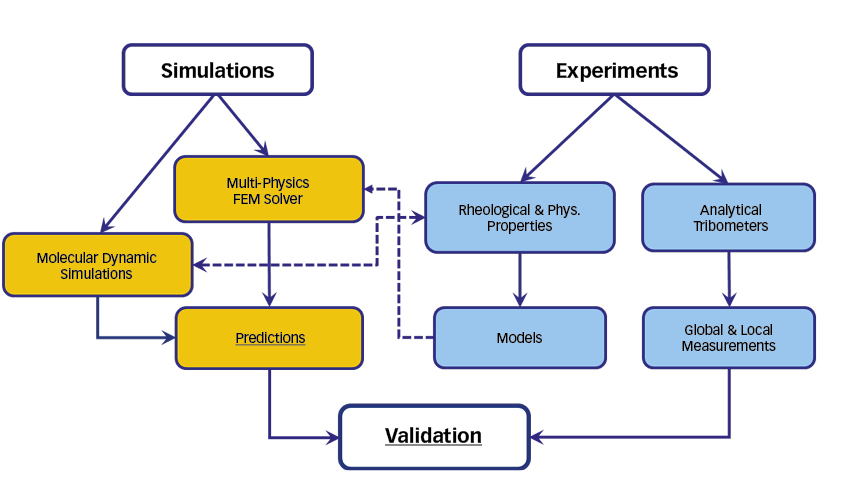
A general description of a quantitative multi-physics, multi-scale and multi-approach to lubrication and lubricants
The chart in fig. 1 summarizes this multi-approach. Tribology and thus lubrication are by nature multidisciplinary fields. This naturally means that it is necessary to consider that several disciplines can interact, but perhaps the most important point is to be able to conduct simultaneously experimental research and numerical modelling and simulation.
The right-hand side of fig. 1 is divided into two contributions for the sake of clarity. The first is tribometry, which implies metrology of the quantities relative to tribology (friction, film thickness, pressure, temperature, etc.), and metrology, meaning accurate calibration, appropriate uncertainties and repeatability. The second contribution should be an essential part of any research in lubrication: it concerns the physical characteristics of the lubricant and, above all, its rheological properties. After all, viscosity and density of the lubricant are two quantities explicitly present in the Reynolds equation, so they are important parameters to consider and characterize in a proper way. For viscosity this seems trivial, but the film thickness at the centre of an elastohydrodynamic (EHD) contact is also directly dependent on the pressure influence on the lubricant’s density. In addition, solving any thermal problem requires the knowledge, and thus the characterization, of the lubricant’s conductivity and specific heat. It should be underlined that these last parameters vary substantially with pressure and temperature, and we have shown that these variations can have a significant influence on friction [1]. After completion of these experimental characterizations, it is necessary to represent the variations of the measured quantities by robust models, established on a physical basis and not on simple mathematical formulas or regressions. The latter can allow interpolation but not extrapolation of values that are beyond the areas covered by the experiment and that may not cover the ranges found in EHL. This is not only important for assessing whether the lubricant properties are suitable over a wide range of conditions, but it is a fundamental and required contribution for conducting quantitative simulations.
The left-hand side of fig. 1 presents the numerical approach. On the far left is molecular dynamic simulation, based on atomistic considerations and allowing two types of results to be obtained at the nanometre scale, which is also the scale of the numerical models. On the one hand, it is possible to obtain “in silico” the physical state and transport properties of a fluid under imposed pressure and temperature conditions. From this point of view, molecular dynamic simulations can provide useful complements to experiments aimed at establishing the physical characteristics of a lubricant. On the other hand, this type of computational approach makes it possible to study the response of highly confined fluid films and their interfaces with solid media at spatial scales where the continuous mechanics approach is no longer valid. This last approach is the foundation of the multi-physics solver, based here on the finite element method (fig. 1). This tool enables prediction of film thickness and friction in EHD contacts for all types of geometry (linear, circular, elliptical, torus/plane) and kinematics (rolling, sliding, spinning, skew). The actual behaviour of the lubricant is taken into account, and the solver considers heat dissipation. In addition to the operating conditions, the input data are the rheological and physical properties of the lubricant through the models mentioned before.
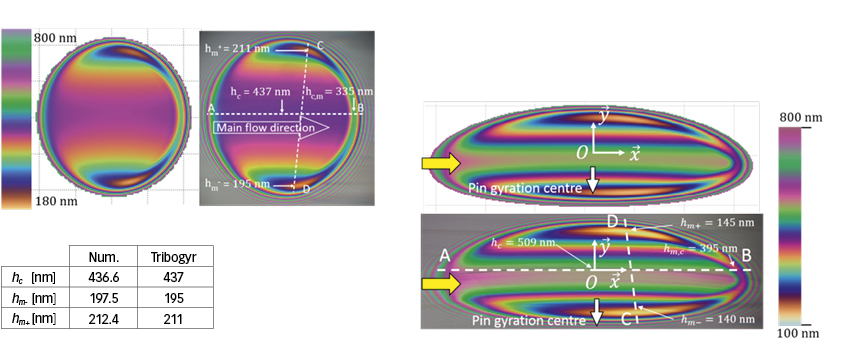
The excellent agreement between experiments and the numerical model is directly included in fig. 2 (left) for the ball-on-plane configuration, indicating a maximum deviation of 1 % whatever the location where the film thickness was estimated. The agreement is also very good in the torus-on-plane case where deviations of 1.5 % were observed on the curved contact central line and of 5 % in the minimum film thickness regions.
Some highlights from the works conducted at LaMCoS-INSA Lyon within the research chair entitled “Lubricated Interfaces for the Future” are summarized hereafter. The title chosen for this cooperation with SKF clearly indicates that the central topic is lubrication, whose main objectives are:
- separation of surfaces to avoid wear and untimely failure of the mechanism by surface damage, and
- friction (linked to energy losses) control via the shearing of a characterized lubricant.
Surface separation and contact integrity
As mentioned above, the dual experimental-modelling approach enables a trustable prediction of lubricant film thickness as soon as the lubricant rheological characterization is coupled with multi-physics models at the contact scale, involving non-Newtonian and thermal effects. The challenge is now to anticipate film thickness formation in the framework of real applications involving poor lubrication conditions, with a potentially spinning/skewing kinematics, and in some cases with no entrainment velocity of the lubricant into the contact. This is the case for full-complement bearings where the contact between two successive rolling elements involves two surfaces moving in opposite directions. In that case, both squeeze and thermal effects (fig. 3) need to be considered to build a predictive film thickness model for zero entrainment velocity contacts, which is crucially lacking in the EHL literature and would greatly help to design those bearings [4].
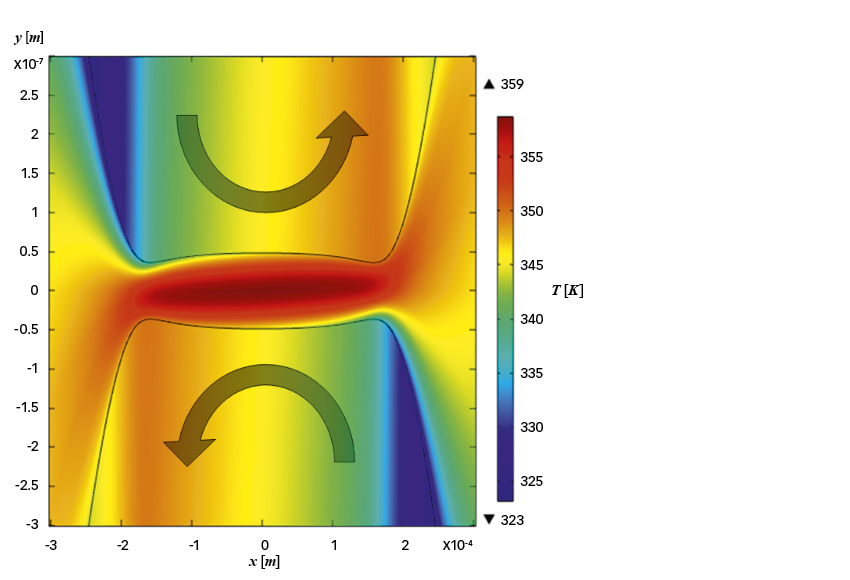
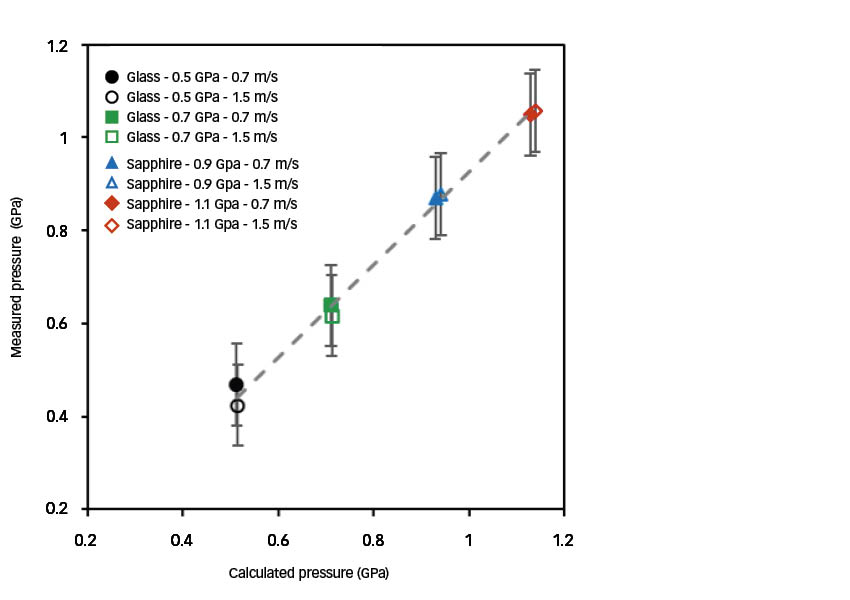
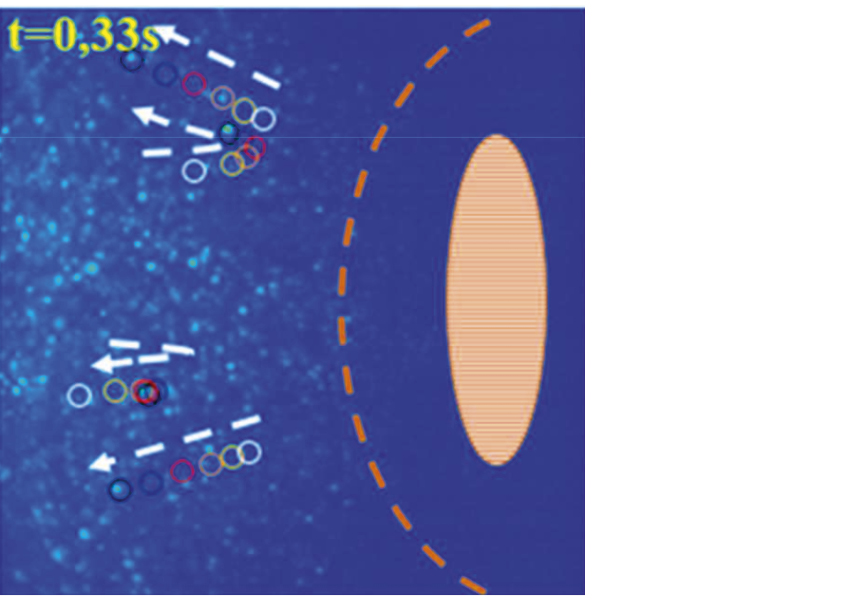
Friction in EHL contacts
Accurate friction prediction of heavily loaded lubricated contacts is still a challenge for tribology in the 21st century. Many physical aspects are intertwined in the frictional response, such that viscous diffusion of the lubricant (Newtonian or non-Newtonian due to shear-thinning effects) is also influenced by thermal effects at high shear and may even be replaced by a plastic-like response (characterized by a limiting shear stress) under high pressure. The joint research chair contributed to this highly important topic in many ways.
Firstly, experimental friction studies of model fluids were led in parallel to physical characterization of the lubricants at various pressure conditions. Brillouin light scattering (BLS) measurements were performed on model lubricants at rest in high-pressure cells under a wide range of pressures and temperatures such as those found in EHD contacts. They highlighted a transition of behaviour associated with the lubricant glass transition [8]. Furthermore, this transition has been correlated with friction measurements performed in a rolling-sliding contact (fig. 6) [9]. In the same figure, the width of the BLS spectra (full width at half maximum, FWHM, in blue) and the lubricant apparent viscosity in the contact (in orange) showed the same transition of behaviour at a mean contact pressure of the order of the lubricant glass transition. To our knowledge, this is the first time such a correlation was supported by experimental results. Finally, the friction plateau has been shown to trigger the limiting shear stress as soon as the maximum pressure in the contact (the Hertzian pressure) reaches the lubricant glass transition pressure [8].
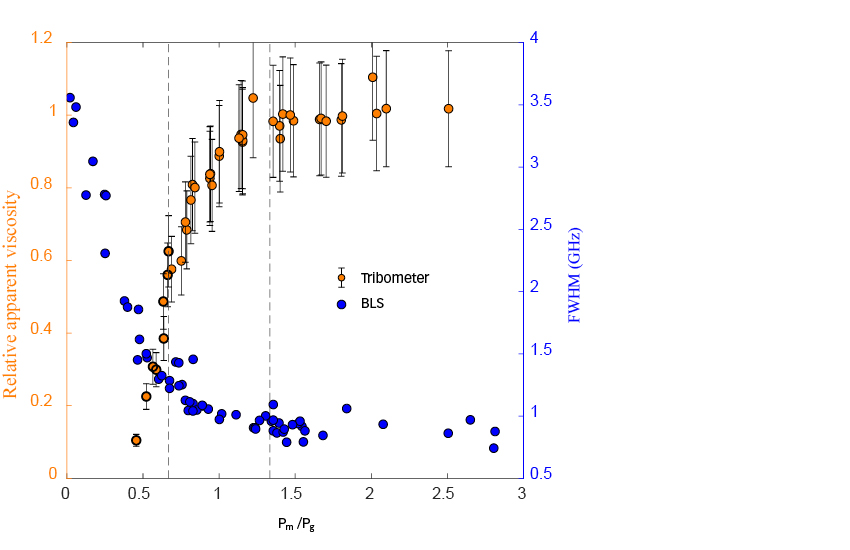
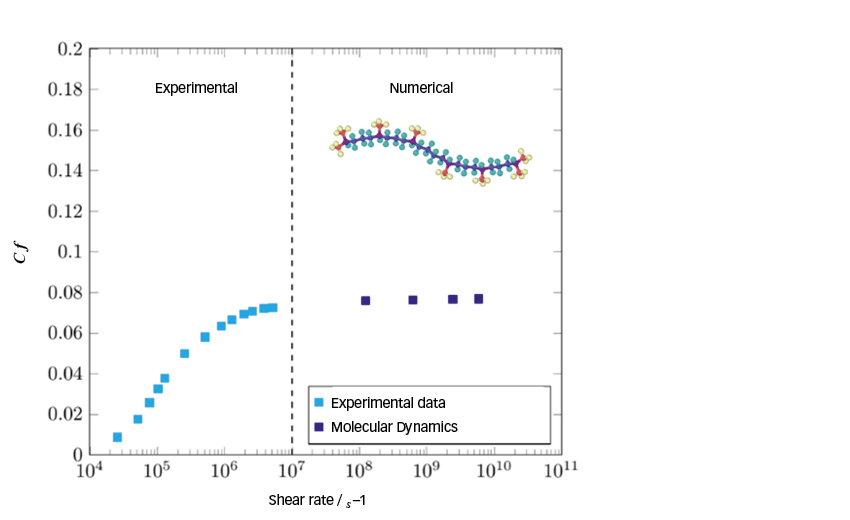
Summary and conclusion
- SKF has developed a collaboration with LaMCoS at INSA Lyon on the topic of the lubrication of large-size spinning contacts starting in the mid-1990s, and then on broader domains covering lubrication in general from the 2000s.
- In May 2013, a research chair funded by SKF and entitled “Lubricated Interfaces for the Future” was launched at LaMCoS – INSA Lyon with the support of the INSA Lyon Foundation and Insavalor, a subsidiary of INSA Lyon for R&D, technology transfer and professional training. The chair was established to find a balance between applied research, which primarily concerns SKF, and more fundamental research that allows university-based researchers to develop new concepts or tools and improve their knowledge, which in turn will be shared with the company’s development engineers and researchers.
- Examples of projects carried out between 2013 and 2019 in the framework of this research chair are described here, showing a representative panel of the research conducted within this specific framework. They are all part of a quantitative multi-physics, multi-scale and multi-approach to lubrication and lubricants.
- The chair, initially launched in May 2013 for a period of six years, was renewed in March 2019 with the same duration.